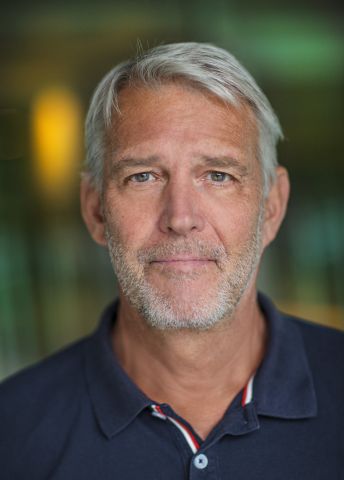
Johan Åqvist
Professor of Theoretical Chemistry
Wallenberg Scholar
Institution:
Uppsala University
Research field:
Computational enzyme design
Wallenberg Scholar
Institution:
Uppsala University
Research field:
Computational enzyme design
Life on Earth flourishes not only in temperate climes with plentiful oxygen and nutrients. If we turn our attention to extreme environments such as active volcanoes or deep-sea trenches, we find there is no shortage of life there either.
Organisms that thrive in places with extreme heat or cold, under high pressure or in acidic environments, are called extremophiles. Many of them are bacteria, but fish can also thrive even where the water temperature approaches zero.
“A feature common to these organisms is that they don’t have any way of regulating their body heat – they have the same internal temperature as their surroundings. The question is how their enzymes and proteins are able to work under these extreme conditions,” says Åqvist, who is a professor of theoretical chemistry at Uppsala University.
The body’s enzymes can be likened to tiny machines that maintain the metabolism of all living cells. But in humans and other mammals enzymes only work under the right conditions. The machinery will stop if the body gets too cold, for instance.
As a Wallenberg Scholar, Åqvist is examining the differences between our enzymes and those of extremophiles. So far he has found surprisingly few.
“Astonishingly, most of the differences we’ve found have been on the surface of the enzyme. Small differences in the surface structure determine whether an enzyme can continue to work under extreme conditions.”
He turns to the computer screen and brings up images of a specific enzyme found in two different bacteria. One of them was obtained from the Arctic Ocean; the other lives in our temperate climate. When he superimposes one structural image over the other, hardly any differences can be seen between them.
“These two enzymes are virtually identical, except for a few surface features. The reason for the difference is that a number of mutations have occurred during evolution, giving the cold-tolerant enzyme new properties.”
The grant enables me to fully understand what gives different enzymes such disparate properties. And I can do this at a department with a blend of expertise and disciplines that creates a very fruitful environment.
Simply put, evolution has softened the surface of the enzyme. The soft surface enables the enzyme to retain its function, or reaction speed, even when the temperature drops to zero.
Enzyme reaction speed is governed by an energy barrier that must be surmounted before the enzyme can catalyze the reactions in a cell. The size of the energy barrier is determined by the amount of energy required for the reaction, and how much order must first be created in the surroundings. The two parameters involved are termed enthalpy and entropy. Enzymes with a softer surface are able to alter the balance between these two parameters so they can continue to function under more extreme conditions.
The discovery was made using large-scale computer simulations of the behavior of selected enzymes under different conditions. The simulations have been made possible by work done in recent decades to map the three-dimensional atomic structure of hundreds of thousands of enzymes. Successful simulation also requires access to the enormous computational power of NAISS – the National Academic Infrastructure for Supercomputing in Sweden.
“Large-scale simulation enables us to calculate everything from enzyme reaction speed and binding strength to activation energy and melting temperature,” Åqvist says.
The simulations enable the scientists to predict which mutations form the basis for enzyme adaptability. The results of the computations are then confirmed by biochemical experiments in the laboratory.
“For the first time we can explain how various mutations impact enzyme adaptability. And we are among the first in the world to do so by simulating reactions using large-scale computations. This approach is much faster than existing experimental methods,” says Åqvist.
The field of computational enzyme design has grown enormously in recent years, and is now a hot research topic. The ability to create enzymes with new characteristics is vital, especially in the pharmaceutical industry, which needs new ways of developing drugs with greater precision and in a shorter time.
Tailored enzymes have potential for numerous applications – from manufacturing biofuels to breaking down toxins in the environment. A more mundane example is using them to make detergents that are equally effective in cold and hot water.
Åqvist was one of the first people in Sweden to develop computational methods in the field of molecular dynamics. As far back as the 1980s, when he was working on his doctoral thesis, he developed methods to calculate enzyme reaction speed on the basis of their three-dimensional structure. He continues to be driven in equal parts by curiosity and a desire to contribute to the development of new technologies.
“I want to understand what evolution has done to make this work. I also want to contribute to the possibility of designing enzymes with unique properties.”
Text Magnus Trogen Pahlén
Translation Maxwell Arding
Photo Magnus Bergström